Marcelo Alcantara Holanda +
Renata dos Santos Vasconcelos +
Betina Santos Tomaz +
By the end of this chapter, the reader should be able to:
-
Understand the concept of patient-ventilator asynchrony
-
Understand its physiological and clinical effects
-
Recognize the types and mechanisms involved
-
Know the main treatment approaches
- Concept and general aspects
- Physiological and clinical effects
- Classification of asynchronies
- References
-
Holanda MA, Vasconcelos RDS, Ferreira JC, Pinheiro BV. Patient-ventilator asynchrony. J Bras Pneumol. 2018 Jul-Aug;44(4):321-333.
-
Bruni A, Garofalo E, Pelaia C, Messina A, Cammarota G, Murabito P, Corrado S, Vetrugno L, Longhini F, Navalesi P. Patient ventilator asynchrony in adult critically ill patients. Minerva Anestesiol., v. 85, n. 6, p: 676-688, 2019.
-
Bulleri E, Fusi C, Bambi S, Pisani L. Patient-ventilator asynchronies: types, outcomes and nursing detection skills. Acta Biomed. 2018 Dec 7;89(7-S):6-18.
-
Damiani LF, Bruhn A, Retamal J, Bugedo G. Patient-ventilator dyssynchronies: Are they all the same? A clinical classification to guide actions. J Crit Care. 2020 Dec;60:50-57.
-
Garofalo E, Bruni A, Pelaia C, Liparota L, Lombardo N, Longhini F, Navalesi P. Recognizing, quantifying and managing patient-ventilator asynchrony in invasive and noninvasive ventilation. Expert Rev Respir Med., n. 12, v. 7, p:557-567, 2018.
-
Netherlands, Marcelo Alcantara et al. Patient-ventilator asynchrony. Brazilian Journal of Pulmonology [online]. 2018, v. 44, n. 4, pp. 321-333.
-
Ramirez II, Arellano DH, Adasme RS, Landeros JM, Salinas FA, Vargas AG, Vasquez FJ, Lobos IA, Oyarzun ML, Restrepo RD. Ability of ICU Health-Care Professionals to Identify Patient-Ventilator Asynchrony Using Waveform Analysis. Respir Care., v. 62; n. 2, p:144-149, 2017.
-
Sousa MLA, Magrans R, Hayashi FK, Blanch L, Kacmarek RM, Ferreira JC. EPISYNC study: predictors of patient-ventilator asynchrony in a prospective cohort of patients under invasive mechanical ventilation - study protocol. BMJ Open. 2019 May 22;9(5):e028601.
-
Telias I, Beitler JR. Reverse Triggering, the Rhythm Dyssynchrony: Potential Implications for Lung and Diaphragm Protection. Am J Respir Crit Care Med. 2021 Jan 1;203(1):5-6.
-
Vasconcelos RS, Melo LH, Sales RP, Marinho LS, Deulefeu FC, Reis RC, Alves-de-Almeida M, Holanda MA. Effect of an automatic triggering and cycling system on comfort and patient-ventilator synchrony during pressure support ventilation. Respiration, v. 86, n. 6, p: 497-503, 2013.
-
Vasconcelos RS, Sales RP, Melo LHP, Marinho LS, Bastos VP, Nogueira ADN, Ferreira JC, Holanda MA. Influences of Duration of Inspiratory Effort, Respiratory Mechanics, and Ventilator Type on Asynchrony with Pressure Support and Proportional Assist Ventilation. Respir Care, n. 62, v. 5, p. 550-557, 2017.
-
Wit M. Monitoring of Patient-Ventilator Interaction at the Bedside. Respiratory Care, v. 56, n. 1, p. 61-72, 2011.
Patient-ventilator asynchrony is a mismatch between the patient's pattern of breathing concerning the respiratory demands of time, flow, volume and/or pressure, and the ventilator, in relation to the provision of these parameters. Asynchronous events can range from subtle changes, which require high suspicion and advanced monitoring for their detection, to the apparent "fight" between the patient and the ventilator. Patient-ventilator asynchrony has incidence rates from 10% to 85%. This considerable variation relates to different factors interfering both in its incidence and detection since, in daily clinical practice, its identification is not always easy and its detection at the bedside is deficient and greatly underestimated. In most cases, these phenomena are not clinically perceived, mainly if it occurs when the patient is not agitated. Asynchronies may have causes related to the underlying condition of the patient, limitations or problems with the mechanical ventilator and accessories, or both.
The negative physiological and clinical impact of asynchronies on patient outcomes has been known for about 20 years. However, a central question remains: are asynchronies responsible, that is, are they causally related to worse clinical outcomes, or are they associated phenomena, being just another marker of severity of illness?
Thus, the negative clinical outcomes associated with patient-ventilator asynchronies are the prolongation of MV duration, intensive care unit (ICU) and hospital length of stay, respiratory muscle injury, the need for tracheostomy and increased mortality (figure 1).
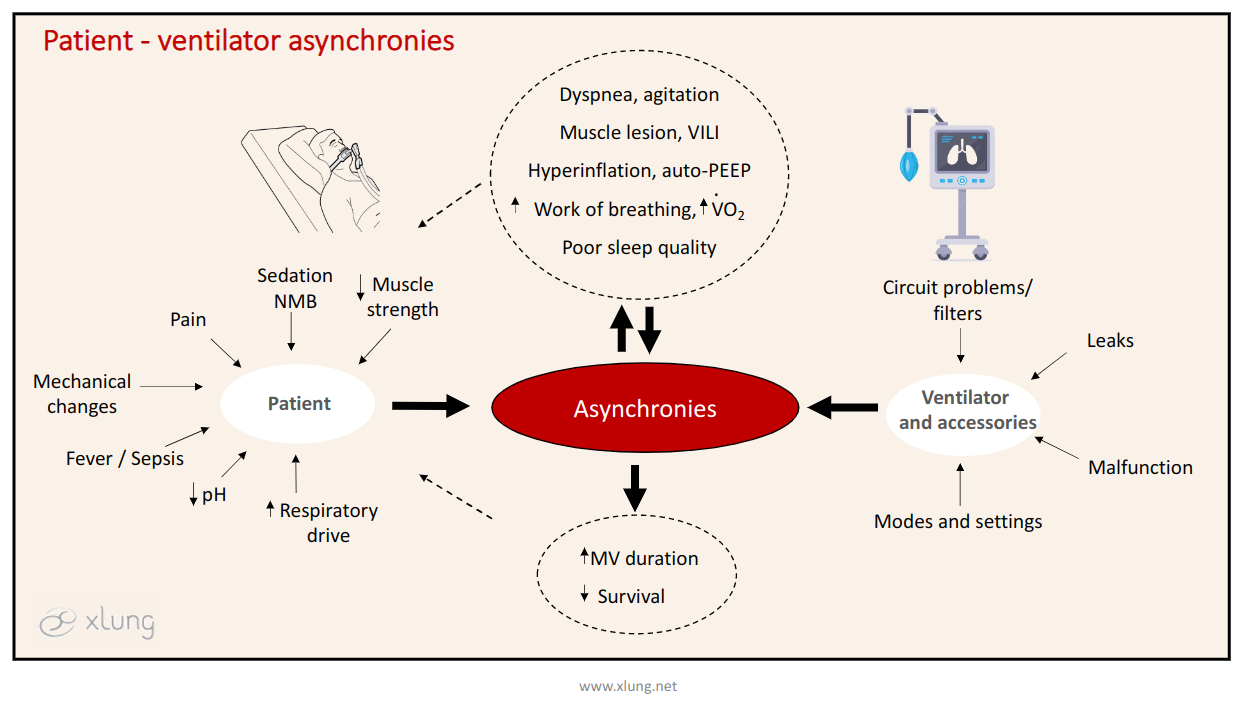
Figure 1: Patient-ventilator asynchrony, determining factors, clinical and physiological effects, and associated outcomes. VILI: ventilator-induced lung injury, VO2: volume of oxygen consumed by the tissues, NMB: neuromuscular blockade, MV: mechanical ventilation.
It is possible to classify the types of patient-ventilator asynchrony according to the phase of the respiratory cycle in which they occur: at the transition from expiration to inspiration or trigger phase; at the transition from inspiration to expiration, or cycling phase, these two types grouped as phase asynchronies, and during the inspiratory phase itself, characterizing flow asynchronies (figure 2).
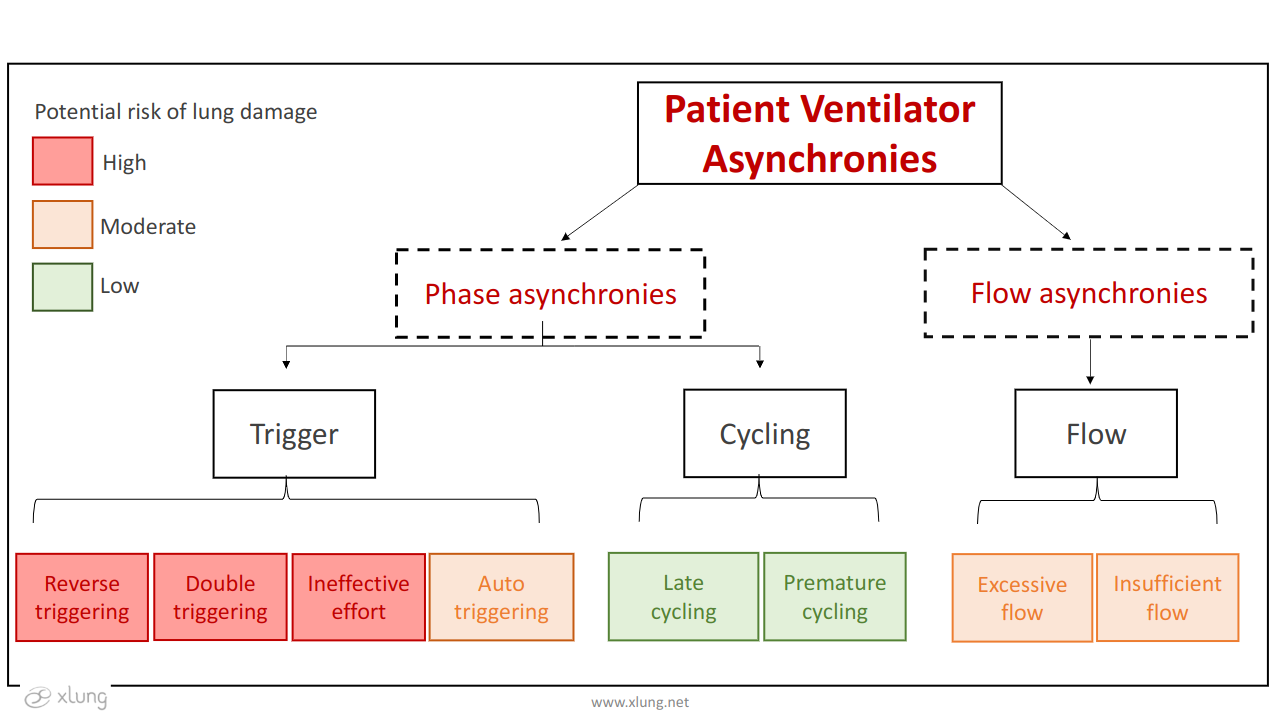
Figure 2: Classification of patient-ventilator asynchronies. Highlight in red, yellow, and green for asynchronies with high, medium, and low risk, respectively, of clinical harm to the patient. Adapted from Daminiani et al, 2020; Holanda et al, 2018.
Triggering asynchronies include ineffective trigger or effort, autotriggering, double-triggering, and reverse triggering. They are named after problems in triggering or initiating the respiratory cycle by the ventilator in response to the patient's effort. The ineffective effort, one of the most common types of asynchrony, consists of the lack of recognition by the mechanical ventilator of the patient's inspiratory muscle effort (figure 3).
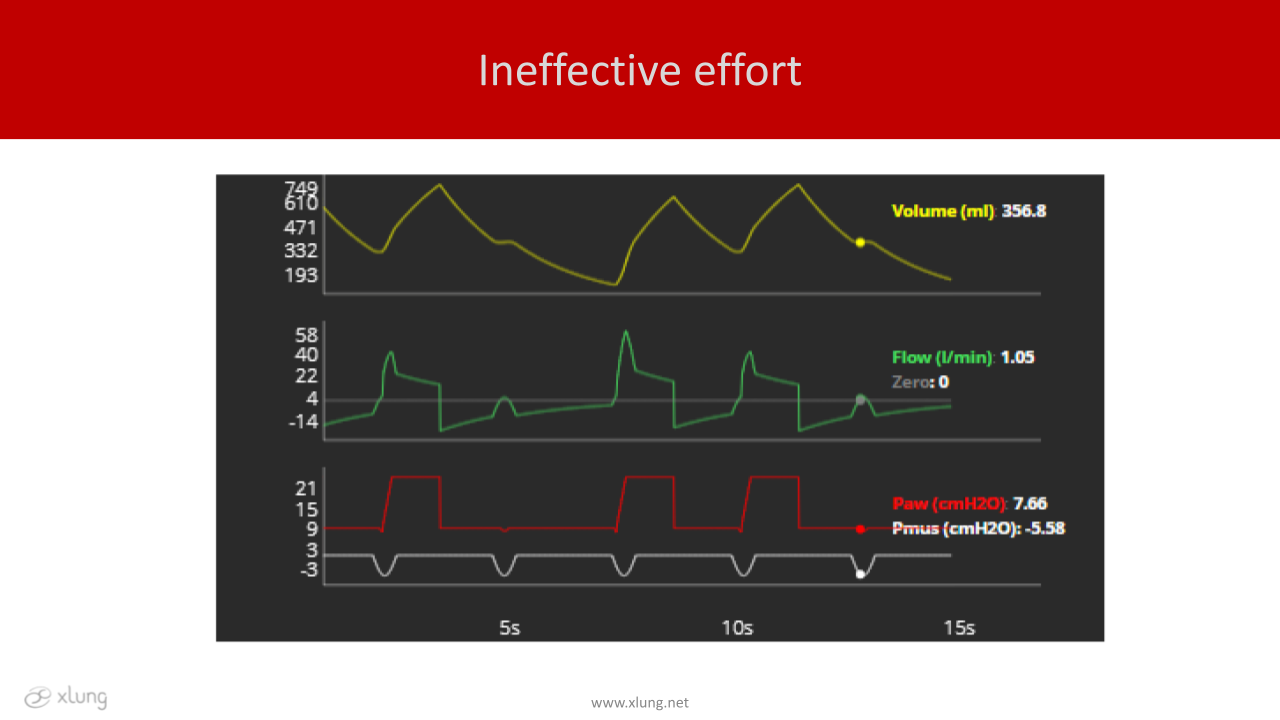
Figure 3. Volume, flow and pressure x time curves, showing a simulation of ineffective effort asynchrony. Patient with airflow obstruction and difficulty triggering due to the presence of auto-PEEP, even with a “physiological” muscular effort, but unable to trigger the ventilator. The dots indicate the moment of an ineffective effort. Paw: airway pressure; Pmus: muscle pressure.
Causes of ineffective efforts may be related to the patient or to the ventilator. In the first case, muscle weakness, blunted respiratory drive due to sedation or neurological problems, including the use of sedatives and neuro-muscular blockers, presence of auto-PEEP with dynamic hyperinflation, especially in patients with COPD. In the second, problems in the ventilator trigger function, inadequate adjustments of this parameter, and obstructions in HME filters, circuits, or tube.
Autotriggering is an asynchrony with the opposite mechanism to the previous one: the ventilator triggers a cycle by improperly recognizing a variation of flow or pressure in the circuit as a spontaneous respiratory muscle effort of the patient. In other words, the sensor system of the device is "deceived" by artifacts, such as leaks, generating depressurization of the circuit or flow and/or pressure oscillations due to the presence of condensate in it, or even by the transmission of intrathoracic pressure variations by the heartbeat and by the ejection of the systolic volume (figures 4 and 5).
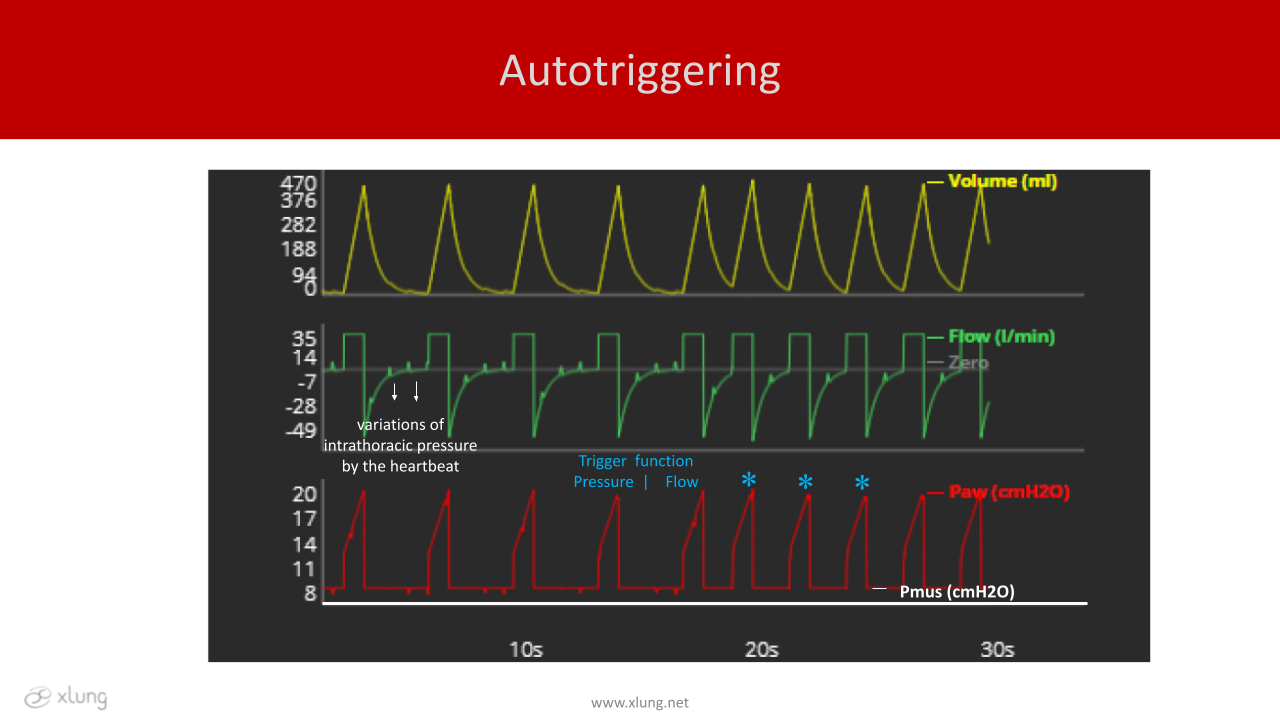
Figure 4. Volume, flow and pressure x time curves, illustrating autotriggering asynchrony. In the simulated situation, there is interference of the heartbeats (HR: 80bpm) during volume-controlled ventilation, with a programmed respiratory rate (RR) of 15 bpm. There are flow and pressure oscillations at regular time intervals according to the HR. The increase in total RR was due to triggers induced by the transmission of heartbeats to the flow wave when the trigger function was switched from pressure to flow. Paw: airway pressure; Pmus: muscle pressure. * autotriggering.
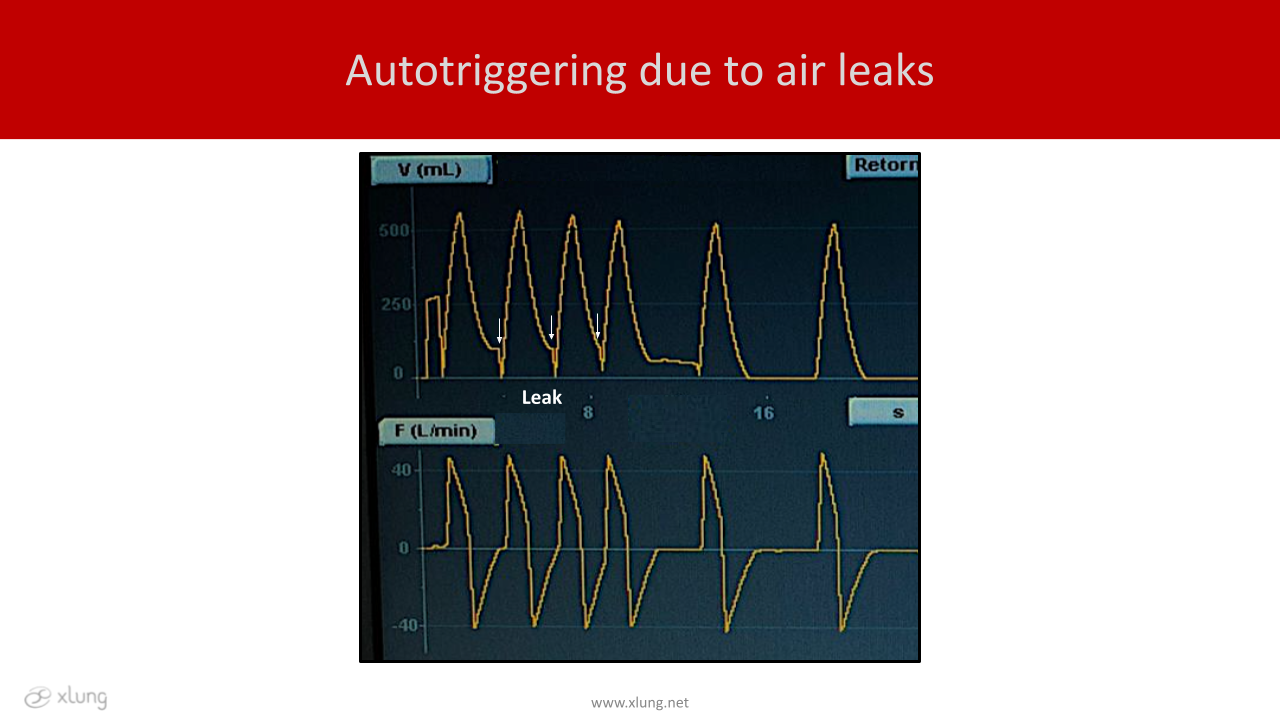
Figure 5. Volume and flow x time curves, illustrating autotriggering due to air leaks. Autotriggering in PSV mode due to leakage (arrows). Trigger function set in 2l/min of flow. The arrows indicate cycles with autotriggering, therefore starting without any relation to the patient’s effort. Observe the tidal volume curve that does not return to the baseline due to the air leak, which, when corrected, resolves the asynchrony in the last breathing cycles.
Double triggering consists of two consecutive cycles delivered by the ventilator for only one single muscle effort of the patient; that is, it occurs when only one respiratory effort of the patient triggers two times in a row. In this case, the patient's inspiratory neural time is longer than the ventilator mechanical cycle. The first trigger results from the patient's effort (figure 6).
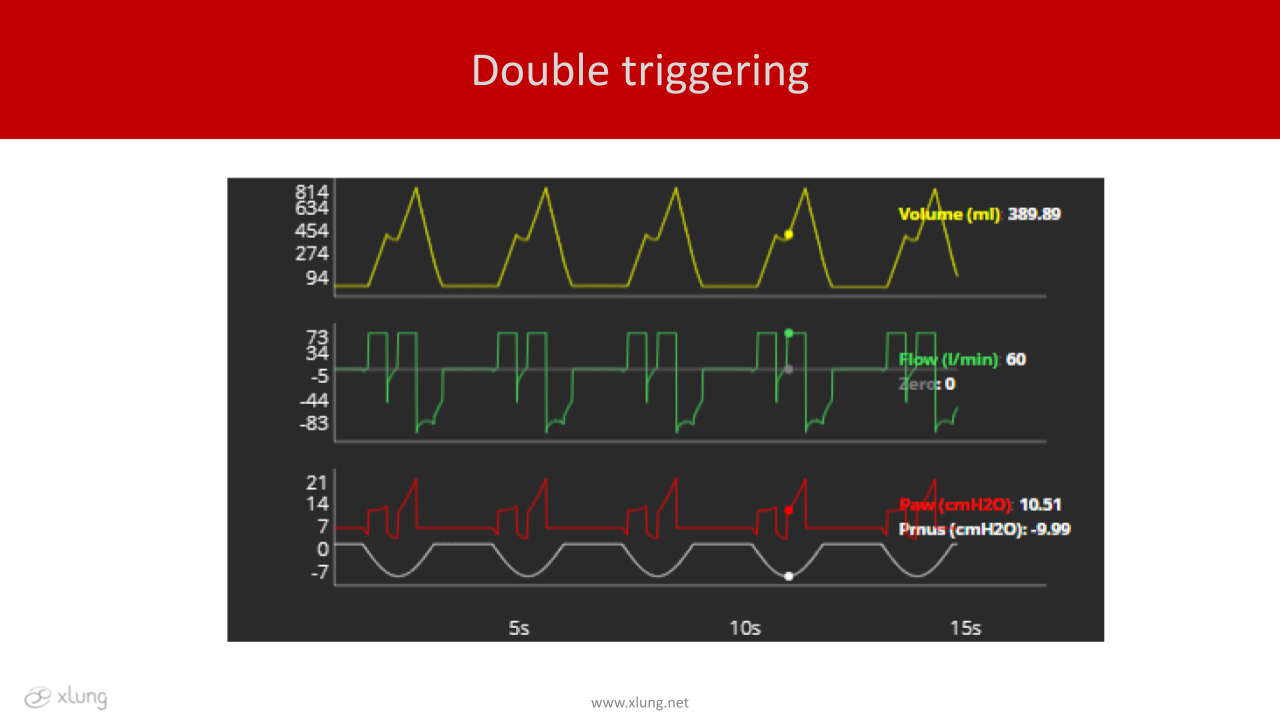
Figure 6. Volume, flow, and pressure x time curves, respectively, illustrating double triggering. In the simulated situation, the double triggering occurs as a result of the patient's neural time being longer than the ventilator's mechanical time. Note that the first cycle is always triggered by the patient, in this case, volume-controlled ventilation (VCV) mode. Paw: airway pressure; Pmus: muscle pressure.
Reverse triggering occurs when a patient's effort is triggered by a ventilator-controlled cycle. Therefore, to be classified as such, the patient's effort must happen after and induced by the controlled cycle. This means that the patient is being managed in an assisted-controlled ventilation mode but can also occur in the PSV in cases of autotriggering, that is, a cycle that was not triggered by the patient (figure 7). Sometimes the ventilator-trigged effort does not necessarily result in triggering. We call this type of reverse triggering reverse effort, as no triggering occurs.
Reverse triggering is a specific form of asynchrony, and it has been described in intubated patients under controlled ventilation and seems to be very common in patients receiving sedation. There are several related mechanisms, not yet fully understood, but the literature reports the involvement of spinal reflexes, and hiccups (reflex arch), among others.
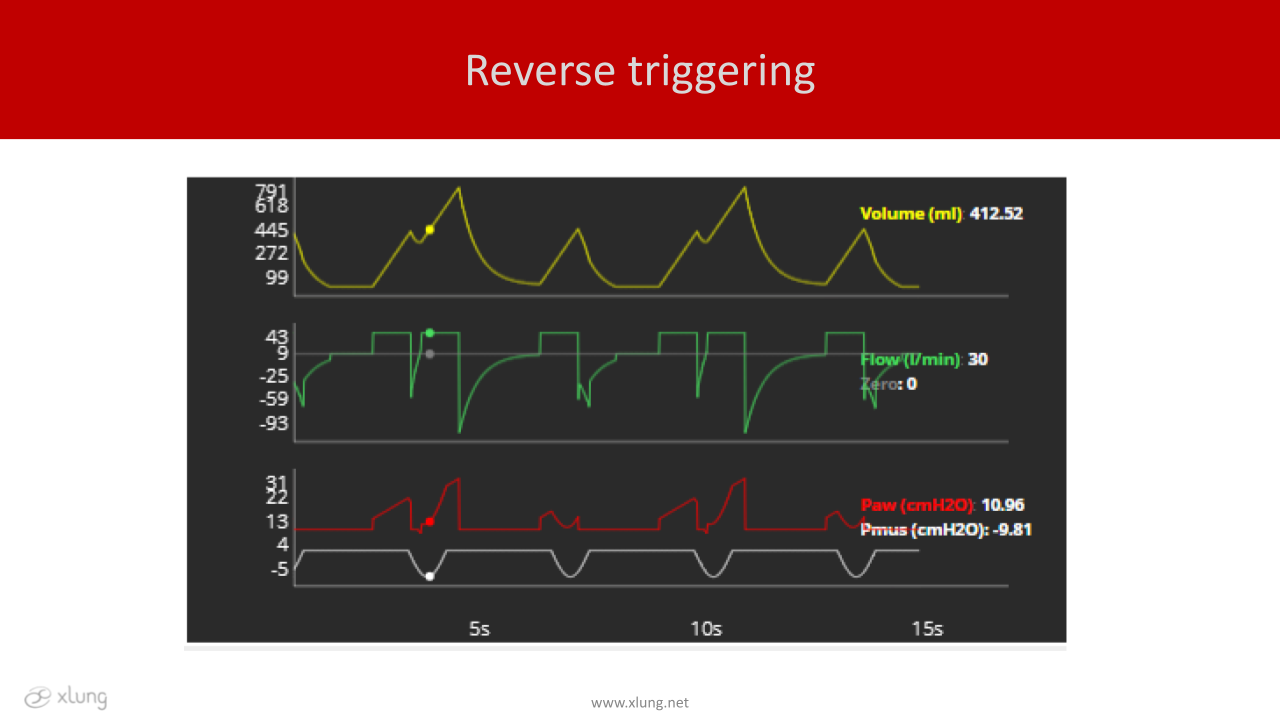
Figure 7. Volume, flow, and pressure x time curves, respectively, illustrating reverse triggering. The reverse triggering corresponds to a respiratory muscle effort triggered by reflex mechanisms resulting from the insufflation of a ventilator-controlled cycle, in this case, in volume-controlled ventilation (VCV) mode. Note the tidal volume stacking (breath stacking) and the elevation of airway pressures during these asynchronies. The dots indicate the moment of reverse triggering. Paw: airway pressure; Pmus: muscle pressure.
In cases of double and reverse triggering, the phenomenon of breath-stacking occurs. This fact is associated with tidal volumes much higher than those set in the ventilator. In cases of patients with ARDS or at risk of this condition, there is a potential for additional lung damage and ventilator-induced lung injury (VILI).
Cycling asynchronies can be premature or late. Premature cycling occurs when the ventilator cycles early; that is, it ends the mechanical inspiratory cycle before the end of the patient's inspiratory muscle effort. In this case, the mechanical inspiratory time is usually less than 50% of the patient's inspiratory time, i.e., the mechanical inspiratory time of the ventilator is much shorter than the patient's neural inspiratory time (figure 8). On the other hand, late cycling occurs when the ventilator offers a mechanical inspiratory time longer than the duration of the patient's muscle effort, usually more than 150% of the patient’s neural inspiratory time. (Figures 8-10).
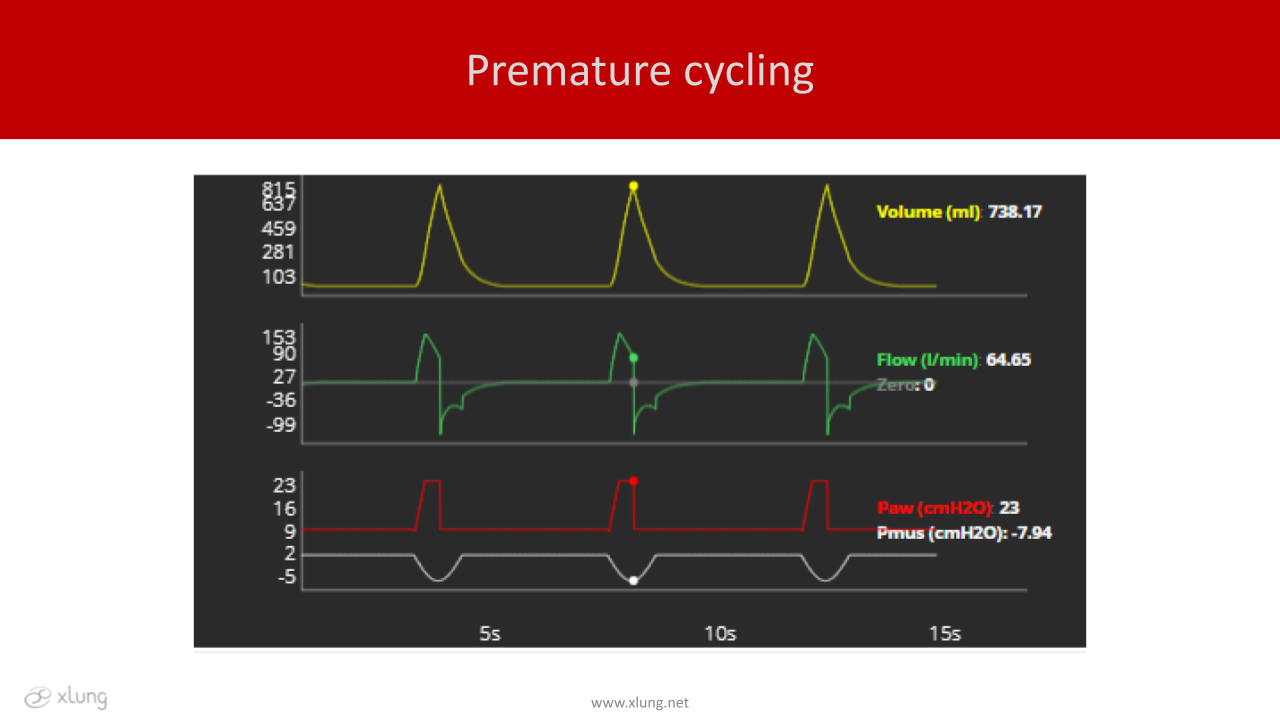
Figure 8: Volume, flow, and pressure x time curves, respectively, illustrating premature cycling asynchrony. The situation above illustrates a patient with restrictive lung disease and cycling asynchrony, which occurs early in relation to the end of the patient's muscular effort. The dots indicate the timing of cycling in a breath during pressure support ventilation (PSV) mode. Paw: airway pressure; Pmus: muscle pressure.
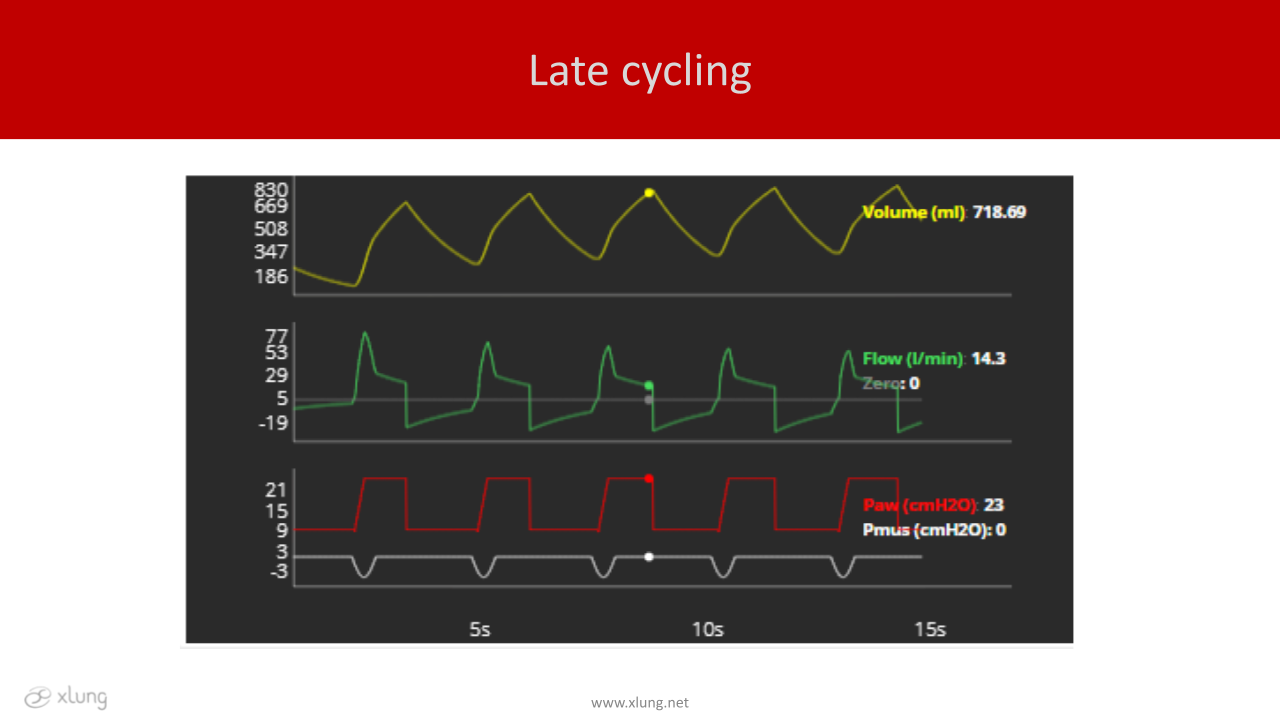
Figure 9: Volume, flow, and pressure x time curves, respectively, illustrating late cycling asynchrony. A patient with COPD is in pressure support ventilation (PSV) mode. Breath cycling occurs late in relation to the end of the patient's muscular effort. The dots indicate the point of delayed timing of cycling. Paw: airway pressure; Pmus: muscle pressure.
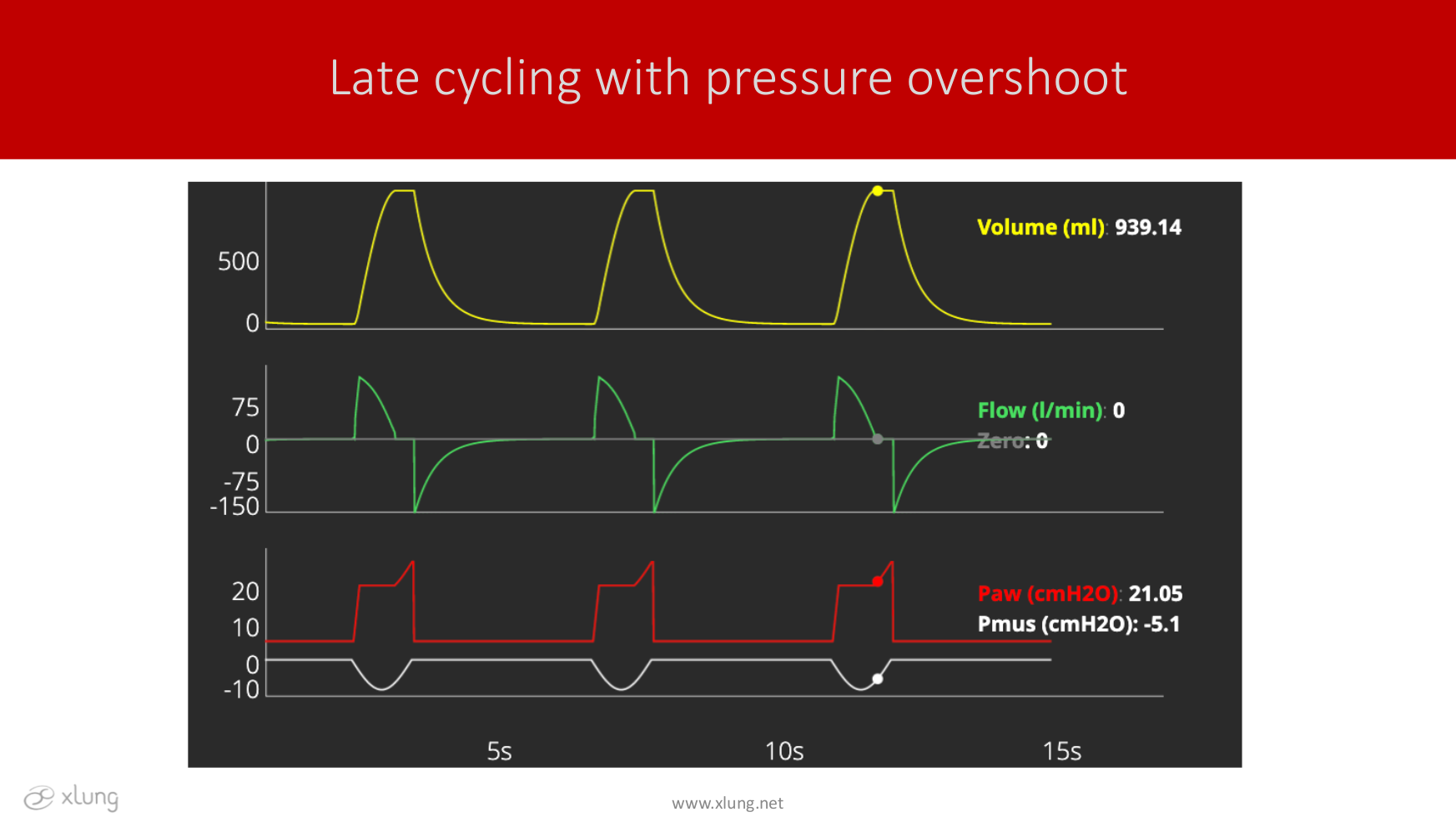
Figure 10: Volume, flow, and pressure x time curves, respectively, illustrating late cycling asynchrony. A patient is in pressure-controlled ventilation (PCV) mode. Pressure overshoot occurs at end expiration with an elevation of airway pressure during muscle relaxation. Airway pressure rises by the effect of a high inspired tidal volume in relation to respiratory system compliance. Paw: airway pressure; Pmus: muscle pressure.
The main causes of cycling asynchronies include poor ventilator adaptation to the patient's neural inspiratory time and characteristics of the respiratory mechanics. For example, patients with COPD have a greater tendency to develop late cycling, and patients with restrictive diseases have a greater predisposition to develop early cycling. As a consequence of this type of asynchronies, these patients may present respiratory distress, airway pressure overshoot, greater need for sedation, and delay in weaning from MV.
Flow asynchronies can be of two types: insufficient or excessive flow. In the first case, the flow received by the patient is lower than his or her ventilatory demand ventilatory demand. Typically occurs in situations where the inspiratory flow is fixed, that is, it is adjusted by the operator and cannot be increased by the spontaneous efforts of the patient, as occurs in the VCV mode. (Figure 11).
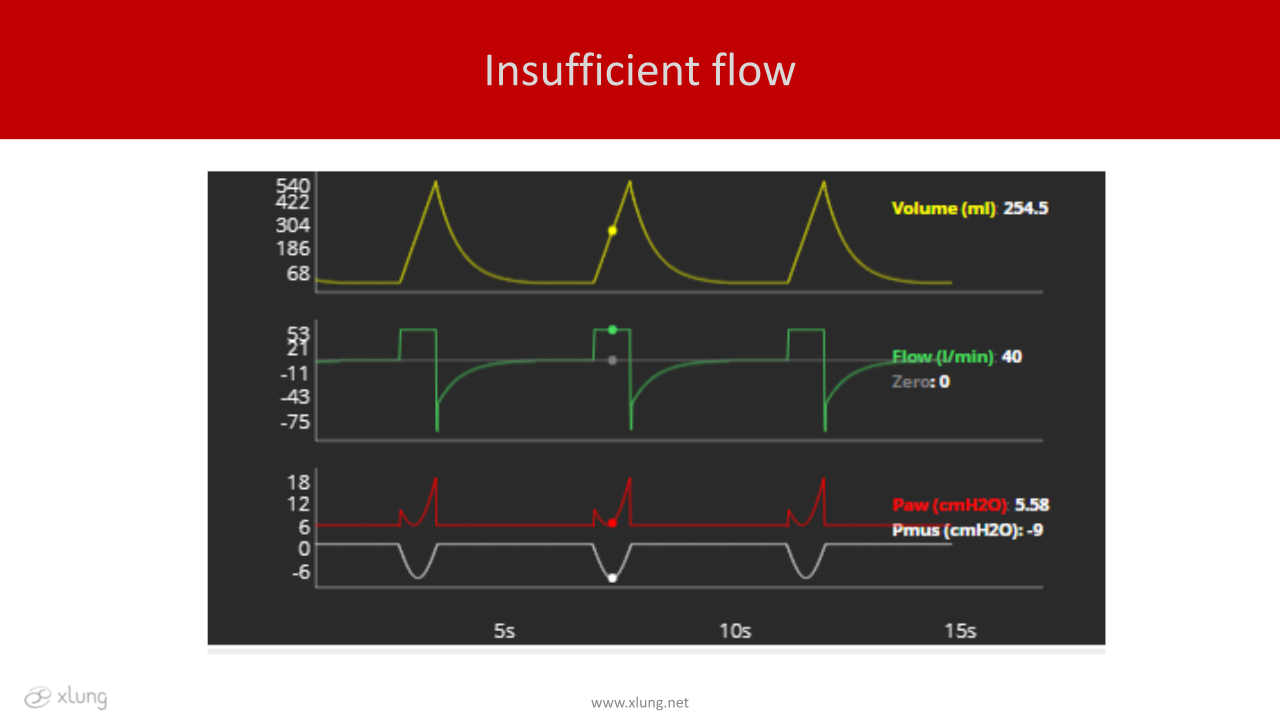
Figure 11: Volume, flow, and pressure x time curves, respectively, illustrating insufficient flow asynchrony. A patient is simulated in volume-controlled ventilation (VCV) mode where the flow is fixed; that is, the flow offer is adjusted by the operator. This situation is commonly called “air hunger”. The dots show the maximal drop in the airway pressure curve in the instant of the maximal negative muscle effort. Paw: airway pressure; Pmus: muscle pressure.
However, it can also occur in the PCV and PSV modalities when the adjustments are insufficient in offering the flow "desired" by the patient. The therapeutic approach may include a focus on reducing the patient's ventilatory demand: correction of hypoxemia, fever, anxiety, pain, acidosis, etc., and/or increasing the flow supply by doing appropriate settings to each mode, observing the comfort and the use of the accessory muscles by the patient, as well as the conformation of the pressure-time curves. And yet, when in VCV mode, a switch to PCV or PSV modes, which have a flow profile more responsive to the patient’s demand, can be a good alternative. In addition, in these modes, the rise time setting directly influences the initial flow delivered immediately after triggering the ventilator. The shorter the rise time, the faster the flow and the initial pressurization of the circuit will be. A shorter ascent or rise time is recommended in patients with clinical signs of "air hunger". In this sense, some ventilators have a resource called adaptative flow (also called demand flow) in the VCV mode, where the ventilator, based on the muscle effort, increases the flow rate to meet the patient's ventilatory demand.
Overflow asynchrony occurs due to the exaggerated supply of inspiratory flow. In some cases, excessive pressurization may occur, characterizing a flow inlet overshoot in PCV or PSV modes. The best option is to reduce the flow supply by reducing the programmed value, in VCV mode, and reduction of the pressure values applied above PEEP and/or increase or prolong the rise time, in PCV and PSV modes (figure 12).
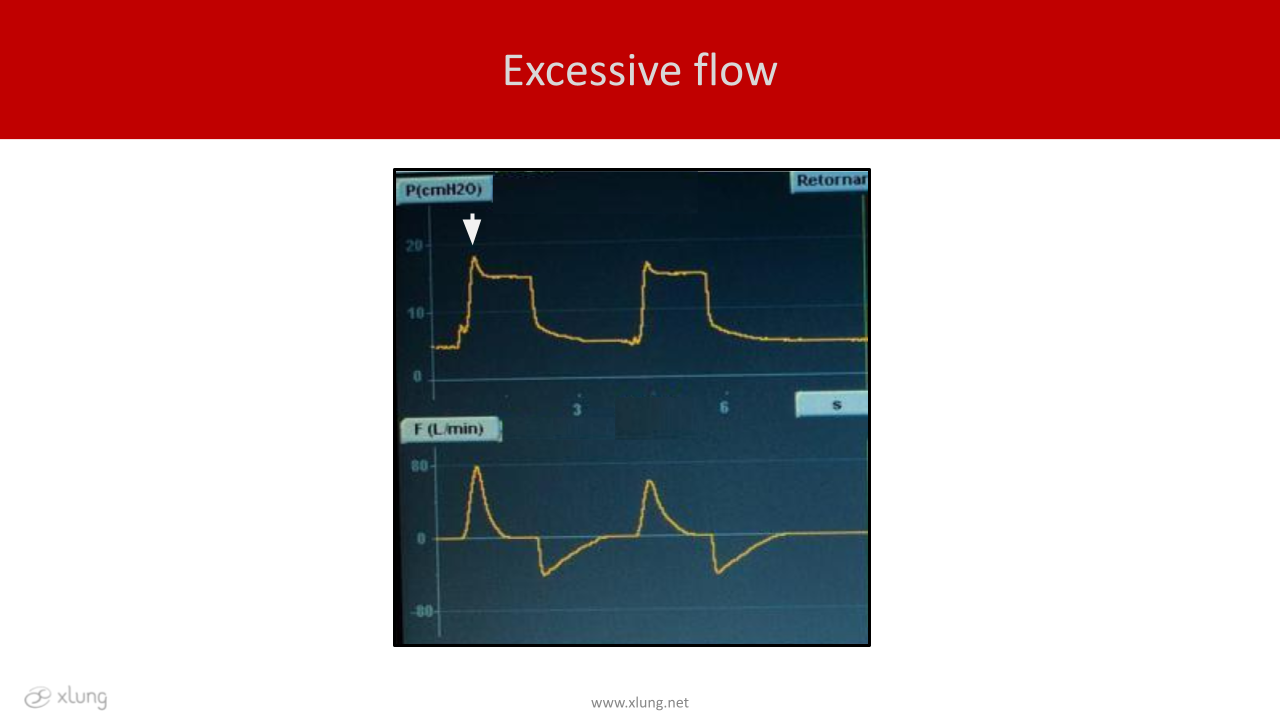
Figure 12. Pressure and flow x time curves, respectively, illustrating excessive flow type asynchrony. A patient is in pressure-controlled ventilation (PCV) mode Excessive initial flow is associated with an early pressure overshoot (white arrow) at the beginning of the respiratory cycle.
Tables 1 and 2 summarize the main mechanisms, risks, and possible solutions of the patient-ventilator asynchronies. Although the use of medications (sedatives) has been reported as a therapeutic strategy in some cases, this option should not be used as a treatment of choice. The best recommendation is that this approach should only be used after the optimization of ventilatory parameters, given the impact of excessive sedation on the patient's outcomes. It is important to remember that the approach to solving asynchronies involves comprehensive care that includes other influencing factors, such as evaluation of the patient's underlying disease and its clinical evolution, multidisciplinary assistant team care, medications in use, and others.
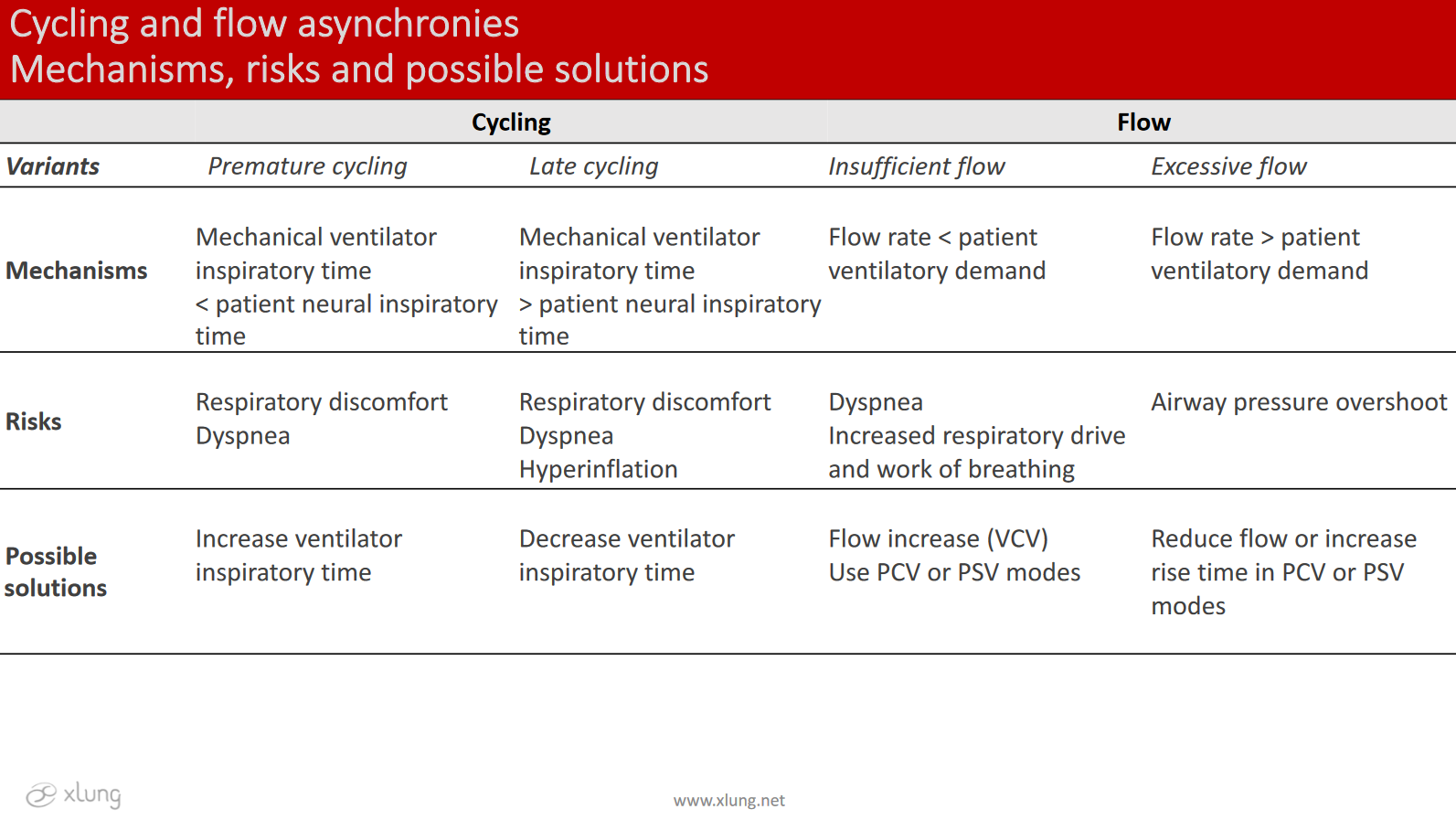
Table 2. Main mechanisms, risks, and possible solutions of cycling and flow asynchronies. PCV: Pressure-controlled ventilation PSV: Pressure-support ventilation
Figures 13 and 14 show the main changes observed in the curves of respiratory mechanics (volume, flow, and pressure) displayed on the mechanical ventilator screen for the identification of asynchronies, with the exception of Pmus which is not routinely visualized.
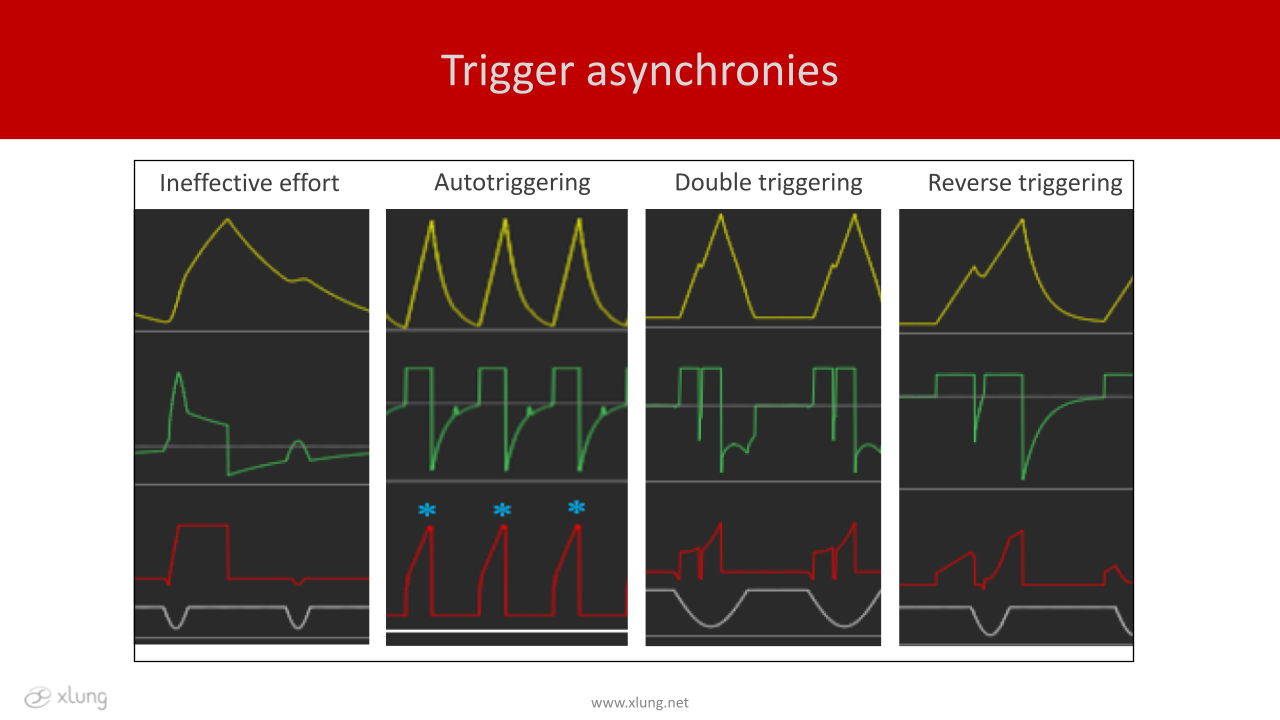
Figure 13. Volume, flow and pressure x time curves displayed on the mechanical ventilator screen. Yellow: volume (ml); green: flow (l/min); red: pressure (cmH2O), gray: muscle pressure (cmH2O).*autotriggering.
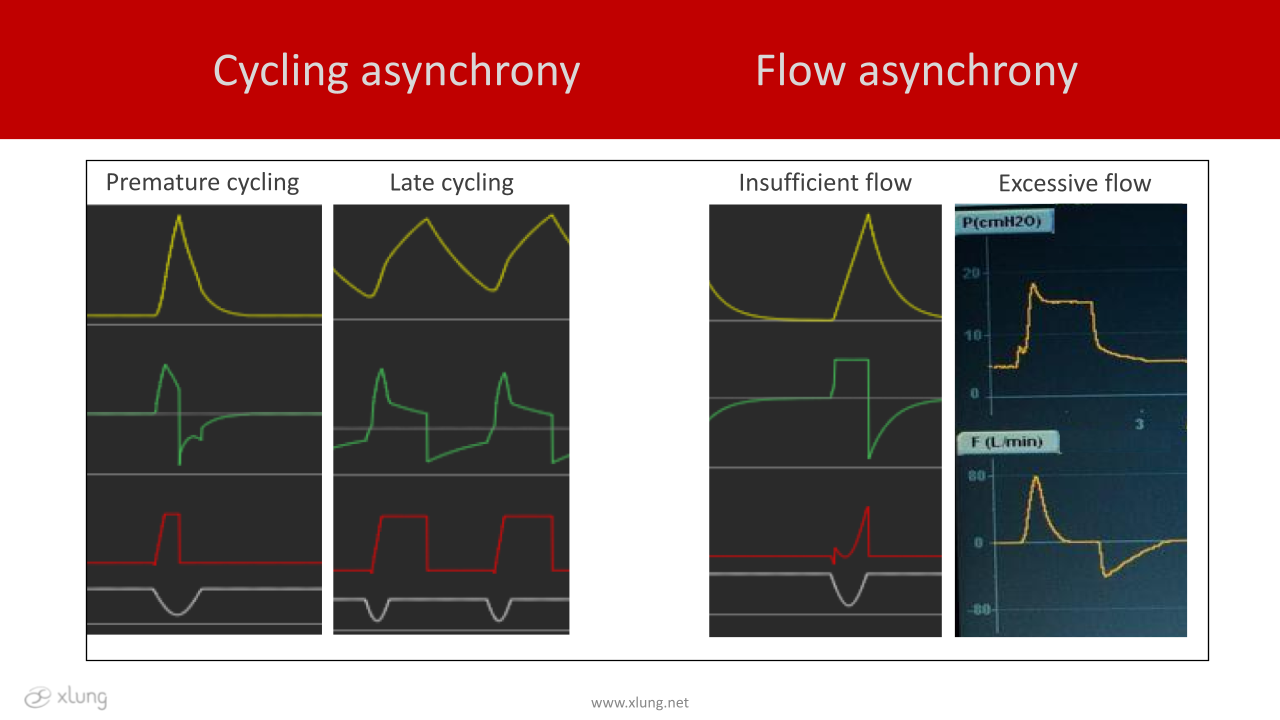
Figure 14. Volume, flow and pressure x time curves displayed on the mechanical ventilator screen. Yellow: volume (ml); green: flow (l/min); red: pressure (cmH2O), gray: muscle pressure (cmH2O). Excessive flow curves are both yellow, in the top, pressure, and in the bottom, flow x time.
There are several tools to evaluate the neural time of the patient to analyze patient-ventilator asynchronies, such as measurement of diaphragmatic electrical activity by electromyography (EMG) and monitoring of esophageal pressure (Pes), both considered ideal methods for the detection of this phenomenon. However, they require invasive and sophisticated, and/or costly procedures, so their availability in daily clinical practice is limited. In this context, the curves of respiratory flow, volume, and pressure x time, displayed on the mechanical ventilator, have been reported as useful tools in the identification of patient-ventilator asynchronies in daily practice. In addition to being considered a noninvasive and reliable alternative, this approach also shows a correlation with methods using Pes or diaphragm EMG. To carry out such an assessment it is important to follow some recommendations (table 3).
New perspectives in the diagnosis and treatment of asynchronies have gained prominence in recent years, including new ventilatory modes, such as NAVA (Neurally - Adjusted Ventilatory Assist) and PAV+ (proportional assist ventilation plus), as well as dedicated softwares for the automated detection and quantification of the main asynchronies. Although promising, they are still little accessible and have been more commonly used in research. Training programs in the asynchrony detection and treatment should be stimulated and disseminated on a large scale, including the use of virtual and realistic simulations for this purpose.